In the previous chapter, we saw what selective pressure is. Let us now look at a few examples of the effects that the combination of mutations and natural selection has in nature.
Example of Mutations and Natural Selection in Natural Populations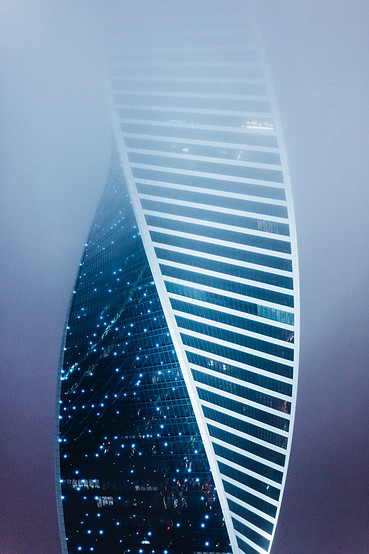
Example: butterflies, bacteria, and the speed of evolution. Bacteria from the genus Wolbachia parasitize insects and are among the most successful organisms on the planet Earth in terms of number and distribution. Several species of these bacteria live in butterflies, where they have developed a very effective way to speed up their transmission.
Namely, the eggs laid by infected female butterflies also carry infection. Male sperm, however, do not transmit the infection, and the bacteria that infect males never manage to leave offspring. When the male butterfly dies, the bacteria die along with it.
Hence, these types of bacteria have evolved into poisonous proteins. In the reproductive organs of an infected female, bacteria selectively kill eggs from this protein from which males would otherwise hatch, leaving the eggs with the females intact. This process leads to a situation where very few males experience hatching from an egg, and the butterfly population consists almost entirely of females (almost all infected with Wolbachia).
The species of widespread tropical butterfly Hypolimnas Bolina was a fine example of this. On the Samoan island of Savai, females of this butterfly were common, while males made up less than 1% of the total population. But in 2005, something new happened: the butterfly evolved a new gene, a suppressor, which disabled the action of the bacterial toxin. Within just one year, males of this species suddenly became so common that they made up 39% of the total population on the island.
Mutations and Natural Selection Produces New Information
This is, of course, an obvious result of the processes we discussed in previous chapters. Mutations produced new information; new information came under strong selective pressure when bacteria exerted it on butterflies, and from there, it suddenly spread through the population.
This fact points us to one important principle: new selective pressures quickly change the population’s genetic composition. This is a point worth further clarifying.
Let’s take another documented example: mice (Chaetodipus Intermedius) that settled in an area that is mostly covered with volcanic ash. Mice with pale fur color are clearly visible on a dark background and represent easy prey for predators. This produces strong pressure for darker fur.
And indeed, these mice quickly developed darker and darker fur until they eventually turned black, a color very similar to the color of the sand in which they live. This is now the limit of adaptation: if fur fits in with the sand, it can no longer evolve to an “even better” fit with the sand (there is no better, “black-brown” color). Mutations will continue to produce fur color variations in the future, but all colors except this one will be eliminated by natural selection; therefore, the color of the fur will not change further.
Mutations and Natural Selection Are Not a Quick Process
This brings us to the reason for the relative stability of many species’ traits. Once a species adapts to a change in the environment, that adaptation remains stable until some new change produces new selective pressure.
Hence, organisms living in stable conditions (so-called stable ecological niches) will change very slowly (examples of this are deep-sea crabs, whose environment has not changed for hundreds of millions of years; today’s crabs are very similar, though not identical, to species that are existed hundreds of millions of years ago). On the other hand, organisms that live in changing environments will change much faster.
We can now ask one fundamental question here: what are the probabilities at stake here? The genomes are large, and the chance of the mutation hitting a specific place is seemingly very small. This is a major stumbling block for many people, which is why it is worth illustrating the time frame and size of the population over which these processes operate. Since today’s examples are very mathematically complex, let’s take one simplified reconstructed version.
Theoretical Example: Mimicry and Butterfly Wings
For many birds, insects are the main source of food, so insects have a strong selective pressure to develop ways to defend themselves against birds. Some have developed toxic or tasteless substances in the body; others have adapted to fit as well as possible into their environment… But there are also some exciting solutions. For example, several species of butterflies have developed spots on their wings that look very much like owl eyes. Small birds that feed on butterflies are terrified of owls, and from there, instead of eating butterflies, they get scared and run away. In the picture above, you can see one such butterfly called Caligo Memnon.
The Mutation Is a Rare Occurrence
But how can something like this happen? The chances of accidentally forming spots that look like owl eyes are minimal, one in ten billion! Certainly, something like that can’t really happen, can it?
In fact, it certainly can if we properly understand the amount of time and the number of individuals involved in the evolutionary process. Let’s consider some average figures.
The usual population of a species of butterfly in a given environment contains about one hundred million individuals. The average butterfly leaves about twenty offspring, which gives us two billion offspring per generation. Consider that each new butterfly carries a hundred new mutations to round off. This is two hundred billion genetic changes in each generation.
If we add a few technical details to this – the size of the average butterfly’s genome (about one hundred million genetic “letters,” i.e., the DNA of base pairs), and the number of genes that control the appearance of wings (about five or six) – we can expect that in giving birth to one hundred and forty thousand individuals in each generation that have a changed appearance of spots on the wings!
We rarely notice this since one hundred and forty thousand of the two billion newborn butterflies are only 0.007% of the total population; therefore, virtually every butterfly we encounter will have a “normal” wing appearance, and only one in every fifteen thousand will have slightly altered spots. If we occasionally come across someone like that, we will think that it is just an unusual specimen (which, in fact, is true).
The Mutation and Natural Selection Occurs in the Long Run
Let us now consider what this means in the context of longer periods of time—one hundred and forty thousand butterflies per generation, one generation per year. This is one hundred and forty million new wing shapes in a thousand years. This is one hundred and forty billion new forms of spots on the wings in a million years.
The absolute largest number – 99.999% – of such changes will have no effect. The new genes will sink into the “background” of the dominant genes within the population, and sooner or later, they will disappear. But this disappearance is not instantaneous. Although in each generation of those 140,000, the majority will die without offspring, there will still be tens of thousands who will leave offspring and leave their characteristics to that offspring. Thus, there will always be a certain part of the species – maybe 0.01% in total – that has significantly different spots on the wings than usual.
And now, one day, one such butterfly has spots that look like an owl’s face in a certain light at a certain angle. This gives the butterfly very little protection – occasionally, maybe once in twenty times, when a bird attacks the offspring of such a butterfly, that bird gets scared. The offspring of this butterfly will only occasionally survive these spots in a situation in which they would not otherwise survive. But this means a selective advantage! This trait will now inevitably spread throughout the population. From generation to generation, more and more butterflies will carry these new traits – thousands, tens of thousands, hundreds of thousands…
New Spots Will Occasionally Have New Mutations That Further Alter the Spots
And as we have seen in previous examples, an increase in the number of individuals that carry a certain trait means that there is an increased chance that that trait will develop further. As this trait spreads throughout the population, the offspring of butterflies with new spots will occasionally have new mutations that further alter the spots. Suppose the spots change to look less like an owl now. In that case, these offspring lose the selective advantage – and the loss of the selective advantage is the same as the negative mutation. It becomes subject to pressure that eliminates those genes from the population.
But if the new additional change produces spots that look even more like an owl and scare the birds even better… that new change now gains an even greater selective advantage and spreads even faster through the population.
Within a few hundred thousand generations, the spots reach the limit of adaptation: they become as similar to owls as possible on the wings of this species of butterfly. Through processes of mutation and selection over a large number of individuals over a long time, we have produced something that seems incredible or impossible at first glance.
Thus, selective pressure controls the development of new traits, determines the speed of their development, and can produce seemingly amazing things over time. But what happens when the existing selective pressure is removed? Let’s look at another example.
Another Example of Mutations and Natural Selection
Example: the eyes of cavefish. In the picture above, you can see one species of cavefish, Amblyopsis Spelaea. One big disadvantage can be seen immediately: this fish (like many related organisms) has no eyes at all.
Within the river fish population, species is an essential trait. Suppose the offspring of a fish give birth to individuals with mutations that cause blindness (which happens relatively often, one fish in every few thousand is born blind). In that case, that offspring will not survive long: it will be easy prey for predators and will be much harder to find food than its fellows. Hence, fish in the river ecosystem is subjected to selective pressure to maintain their species. Since the mutation that destroys vision is strongly negative, it goes against this selective pressure.
In the caves, however, the situation is completely different. Since there is no light, there is no selective pressure to maintain sight: the individuals who see have no advantage over blind individuals.
The eyes of vertebrates expend energy incessantly, even in complete darkness; hence, for a fish in a cave, the eyes represent a constant investment of energy without any gain. If the offspring of a fish is born with mutations that lead to blindness, it has an advantage in this case: it does not constantly invest energy in an unnecessary organ. At the same time, since there is no selective pressure (individuals with vision have no advantage), he did not lose anything.
This is one of many examples of the importance of selective pressure for maintaining existing traits. If selection is removed, there is no creation and dissemination of information, and existing information inevitably degenerates.
So, today we can observe how mutation processes introduce variations and how selection processes change the genetic composition of organisms.
If We Change the Genes Enough, We Will Also Change the Species
Since the genetic composition defines a species, its change automatically means a change of species: if we change the genes enough, we will also change the species. In nature, we can notice different levels of such changes live. For example, horses and donkeys are descendants of the same species divided into two parts; the two parts were then subjected to different selective pressures. The result of accumulated genetic differences is that these two species can no longer produce fertile offspring. The crossing of horses and donkeys produces sterile offspring (mules or mules) that are not able to reproduce further. We can also watch this process live on several natural examples, among which the so-called ring species are perhaps the best.
Example: species and strains of the green nightingale. The green nightingale (Phylloscopus Trochiloides) is a bird that lives in mountain forests and originates from the Himalayan region spreading from its initial area, crossing from mountain to mountain. The population of the green nightingale spread in a ring around the Tibetan steppe (in which there are not enough trees for the nightingale to survive).
During this expansion, however, nightingale populations in separate areas became increasingly distant from each other. Birds from distant mountains accumulated mutations, and populations became more and more diverse. The color and shape of the feathers became significantly different between separate populations.
The nightingale’s song slowly changed along with the move, which led to additional isolation among the populations: the female of one group no longer “understood” the male song of the other group, and thus interbreeding was difficult.
What Is the Overall Effect of These Events?
Neighboring groups are still genetically and visually similar enough to be able to mate. Nightingales from group A regular mate with nightingales from groups F and B; less often with nightingales from groups C and G. However, when the distance increases, there is no more mating: pairs are never established between groups E and A or F and D., And at the very edges of the ring, there is a situation where genetic changes large enough to prevent interbreeding: nightingales from groups G and X cannot mate with each other, because such mating can no longer produce living offspring. Genetic differences are simply too great.
Observing the green nightingale, we can see the mechanism of gradation of species in action: neighboring groups still belong to the same species, but when the distance becomes too great, we must start talking about two species of nightingales.
Species formation through reproductive isolation has also been observed in the laboratory. One concrete example: in 1964, on the ocean coast in California, scientists picked up five or six worms of the species Nereis Acuminata. Of these few worms, these worms (for various experiments in cell biology) have been propagated in thousands of individuals in the laboratory. Shortly afterward, four males and four females were sent to colleagues in a laboratory in the eastern United States, where their offspring continued to live for the next twenty years. During that period, these two populations were isolated from each other.
The End Result of Mutations and Natural Selection Is Reproductive Incompatibility
Twenty years later, the two worm populations have accumulated so many different mutations that they have become reproductively incompatible. Individuals from the eastern group cannot produce offspring at all with individuals from the western group. From one species, two new ones were effectively created.
What is important is that no single mutation produces such results. Changes in species result from the accumulation of several different mutations over many generations under different selective pressures. This brings us to the last essential item:
The individual experiences mutations and the population experiences selection.
In the next chapter, we will summarize all of the above and formulate the rules of evolution.